FAQs
When a new particle physics discovery is made, you may have heard the term “sigma” being used. What does this mean? Why is it so important to talk about sigma when making a claim for a new particle discovery? And why is five sigma in particular so important?
Why does particle physics rely on statistics?
Particles produced in collisions in the Large Hadron Collider (LHC) are tiny and extremely short-lived. Because they almost immediately decay into further particles, it is impossible for physicists to directly “see” them. Instead, they look at the properties of the final particles, such as their charge, mass, spin and velocity. They work like detectives: the end products provide clues to the possible transformations that the particles underwent as they decayed. The probabilities of these so-called “decay channels” are predicted by theory.
In the LHC, millions of particle collisions per second are tracked by the detectors and filtered through trigger systems to identify decays of rare particles. Scientists then analyse the filtered data to look for anomalies, which can indicate new physics.
As with any experiment, there is always a chance of error. Background noise can cause natural fluctuations in the data resulting in statistical error. There is also potential for error if there isn’t enough data, or systematic error caused by faulty equipment or small mistakes in calculations. Scientists look for ways to reduce the impact of these errors to ensure that the claims they make are as accurate as possible.
What is statistical significance?
Imagine rolling a standard die. There is a one in six probability of getting one number. Now imagine rolling two dice – the probability of getting a certain total number varies – there is only one way to roll a two, and six different ways to roll a seven. If you were to roll two dice many, many times and record your results, the shape of the graph would follow a bell-curve known as a normal distribution.
The normal distribution has some interesting properties. It is symmetrical, its peak is called the mean and the data spread is measured using standard deviation. For data that follows a normal distribution, the probability of a data point being within one standard deviation of the mean value is 68%, within two is 95%, within three is even higher.
Standard deviation is represented by the Greek letter σ, or sigma. Measured by numbers of standard deviations from the mean, statistical significance is how far away a certain data point lies from its expected value.
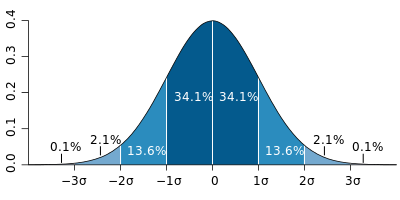
What has this got to do with physics?
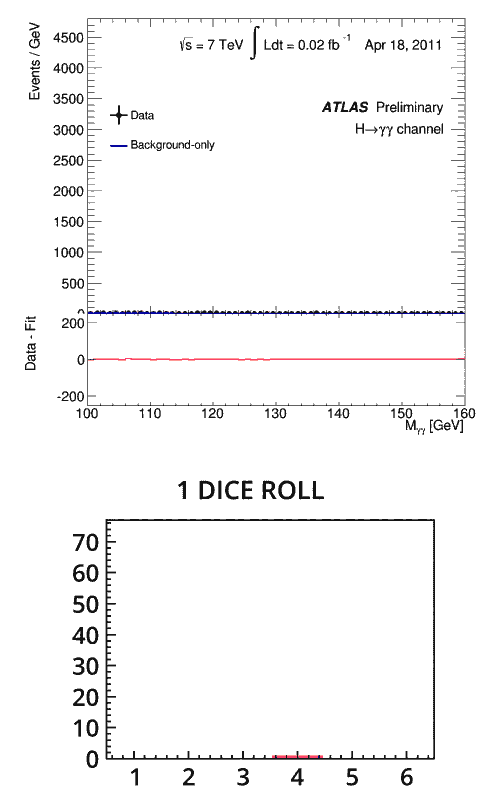
Second image: Animation of the results of 300 dice rolls, where the die has been manipulated to show the number 3 more often than expected. (Image: Piotr Traczyk/CERN)
When scientists record data from the LHC, it is natural that there are small bumps and statistical fluctuations, but these are generally close to the expected value. There is an indication of a new result when there is a larger anomaly. At which point can this anomaly be classified as a new phenomenon? Scientists use statistics to find this out.
Imagine the dice metaphor again. Except this time, you are rolling one die, but you do not know if it is weighted. You roll it once and get a three. There is nothing particularly significant about this – there was a one in six chance of your result – you need more data to determine if it is weighted. You roll it twice, three times, or even more, and every time it lands on a three. At what point can you confirm it is weighted?
There isn’t a particular rule for this, but after around eight times of getting the same number, you’d be pretty certain that it was. The chance of this happening as a fluke is only (1/6)8 = 0.00006%.
In the same way, this is how physicists determine if an anomaly is indeed a result. With more and more data, the likelihood of a statistical fluctuation at a specific point gets smaller and smaller. In the case of the Higgs boson, physicists needed enough data for the statistical significance to pass the threshold of five sigma. Only then could they announce the discovery of “a Higgs-like particle.”
What does it mean when physicists say data has a statistical significance of five sigma?
A result that has a statistical significance of five sigma means the almost certain likelihood that a bump in the data is caused by a new phenomenon, rather than a statistical fluctuation. Scientists calculate this by measuring the signal against the expected fluctuations in the background noise across the whole range. For some results, whose anomalies could lie in either direction above or below the expected value, a significance of five sigma is the 0.00006% chance the data is fluctuation. For other results, like the Higgs boson discovery, a five-sigma significance is the 0.00003% likelihood of a statistical fluctuation, as scientists look for data that exceeds the five-sigma value on one half of the normal distribution graph.
Why is five sigma specifically important for particle physics?
In most areas of science that use statistical analysis, the five-sigma threshold seems overkill. In a population study, such as polls for how people will vote, usually a result with three sigma statistical significance would suffice. However, when discussing the very fabric of the Universe, scientists aim to be as precise as possible. The results of the fundamental nature of matter are high impact and have significant repercussions if they are wrong.
In the past, physicists have noticed results that could indicate new discoveries, with the data having only three to four sigma statistical significance. These have often been disproven as more data is collected.
If there is a systematic error, such as a miscalculation, the high initial significance of five sigma may mean that the results are not completely void. However, this means that the result is not definite and cannot be used to make a claim for a new discovery.
Five sigma is considered the “gold standard” in particle physics because it guarantees an extremely low likelihood of a claim being false.
But not all five sigmas are equal…
Five sigma is generally the accepted value for statistical significance for finding new particles within the Standard Model – those particles that are predicted by theory and lie within our current understanding of nature. Five sigma significance is also accepted when searching for specific properties of particle behaviour, as there is less chance of finding fluctuations elsewhere in the range.
Whether five sigma is enough statistical significance can be determined by comparing the probability of the new hypothesis with the chance it is a statistical fluctuation, taking the theory into account.
For physics beyond the Standard Model, or data that contradicts generally accepted physics, a much higher value of statistical significance is required – effectively enough to “disprove” the previous physics. In his paper “The significance of five sigma,” physicist Louis Lyons suggests that results for more unlikely phenomena should have a higher statistical significance, such as seven sigma for the detection of gravitational waves or the discovery of pentaquarks.
In this paper, Lyons also deems five sigma statistical significance to be enough for the Higgs boson discovery. This is because the theory for the Higgs boson had been predicted, mathematically tested, and generally accepted by the particle physics community well-before the LHC could generate conditions to be able to observe it. But once this was achieved, it still required a high statistical significance to determine if the signal detected was indeed a discovery.
___________________________________________________________________________
A statistical significance of five sigma is rigorous, but it is really a minimum. A higher value for statistical significance cements data as being more reliable. However, achieving results with statistical significance of six, seven, or even eight sigma requires a lot more data, a lot more time, and a lot more energy. In other words, a probability of at most 0.00006% that a new phenomenon is not a statistical fluke is good enough.
Find out more:
- Paper: The significance of five sigma
- Video: The Higgs boson discovery, explained
- Online article: One and two sided probability
The LHC will not generate black holes in the cosmological sense. However, some theories suggest that the formation of tiny 'quantum' black holes may be possible. The observation of such an event would be thrilling in terms of our understanding of the Universe; and would be perfectly safe. More information is available here.
What is the Brout-Englert-Higgs mechanism?
The Brout-Englert-Higgs mechanism (BEH mechanism) describes how fundamental particles get mass. In this theory, developed independently by Robert Brout and François Englert in Belgium and Peter Higgs in the United Kingdom in 1964, fundamental particles acquire mass by interacting with a “field” that permeates the entire Universe. The more strongly the particles interact with the field, the more massive they are.
On the other hand, particles that do not interact with this field do not have mass - for example, the photon. This mechanism is a corner stone of the Standard Model, the theory that describes the elementary particles and forces. Later in 1964, the Americans Gerald Guralnik and Carl Hagen with their British colleague Tom Kibble further contributed to the development of this new idea.
What is the Higgs boson?
The Higgs boson is the quantum particle associated with the Higgs field. Since the field cannot be observed directly, experiments have searched for the particle, whose discovery would prove the existence of the field and confirm the theory.
On 4 July 2012, the ATLAS and CMS collaborations announced the observation of a particle consistent with the long-sought Higgs boson. The analyses performed since then by the two collaborations have confirmed that the particle discovered has the characteristics of the boson described by the theory.
Why is this so important?
At the beginning of the 1970s, physicists realized that there are very close ties between two of the four fundamental forces – the weak force and the electromagnetic force. These two forces can be described within the same unified theory, which forms the basis of the Standard Model. The basic equations of the unified theory correctly describe the two forces in terms of a single electroweak force and its associated force-carrying particles, namely the photon, and the W and Z bosons - except that all of the particles emerge without a mass. While this is true for the photon, we know that the W and Z have large masses, nearly 100 times that of a proton. The Brout-Englert-Higgs mechanism solves this problem by giving a mass to the W and Z bosons. At the same time, within the Standard Model, it also gives masses to other fundamental particles, such as the electron and the quarks.
Is the Higgs mechanism responsible for the mass that is familiar to us?
The Higgs field gives mass only to elementary particles such as electrons and quarks. Quarks form the protons and neutrons in atomic nuclei. Most of the mass of the matter that surrounds - and includes - us comes from these composite protons and neutrons. The quarks inside them account for only a tiny part of their mass, which mainly comes from the strong nuclear force that binds the quarks together. However, without the Higgs field, the Universe would not be the one we know. The elementary particles, such as electrons, would travel at the speed of light, as photons do. They could not be organized into more complex structure like atoms and molecules - and we would not exist.
How long has CERN been looking for the Higgs boson?
The search for the Higgs boson at CERN began in earnest in the late 1980s, with the Large Electron-Positron (LEP) collider, which occupied the tunnel that now houses the Large Hadron Collider (LHC). The experiments at the Tevatron collider at Fermilab in the US also began searching for the Higgs boson in the 1990s. The big difficulty initially was that theory did not predict the mass of the particle and it was possible that it could be found anywhere in a wide range of mass. LEP was shut down in 2000 to make way for the LHC and the LHC experiments took up the search again in 2010.
Is this the end of the quest?
Finding the Higgs boson is not the end of the story; the physicists have to study this particle in detail to measure its properties. Furthermore, many questions remain unanswered. For instance, what is the nature of dark matter, which makes up a large part of the Universe? Why is there far more matter than antimatter in the Universe, when both have been created in equal quantities at the beginning of the Universe? And many other questions...
Were any previous Nobel prizes awarded for work performed at CERN?
Carlo Rubbia and Simon van der Meer won the Nobel prize in physics in 1984 “for their decisive contributions to the large project, which led to the discovery of the field particles W and Z, communicators of weak interaction.”
Georges Charpak won the Nobel prize in physics in 1992 “for his invention and development of particle detectors, in particular the multiwire proportional chamber”. The particle detectors developed by Charpak revolutionized experimental particle physics by increasing considerably the volume of data that can be recorded by detectors.
The Large Hadron Collider (LHC) is the most powerful particle accelerator ever built. The accelerator sits in a tunnel 100 metres underground at CERN, the European Organization for Nuclear Research, on the Franco-Swiss border near Geneva, Switzerland.
What is the LHC?
The LHC is a particle accelerator that pushes protons or ions to near the speed of light. It consists of a 27-kilometre ring of superconducting magnets with a number of accelerating structures that boost the energy of the particles along the way.
Why is it called the “Large Hadron Collider”?
- "Large" refers to its size, approximately 27km in circumference
- "Hadron" because it accelerates protons or ions, which belong to the group of particles called hadrons
- "Collider" because the particles form two beams travelling in opposite directions, which are made to collide at four points around the machine
How does the LHC work?
- The CERN accelerator complex is a succession of machines with increasingly higher energies. Each machine accelerates a beam of particles to a given energy before injecting the beam into the next machine in the chain. This next machine brings the beam to an even higher energy and so on. The LHC is the last element of this chain, in which the beams reach their highest energies.
- Inside the LHC, two particle beams travel at close to the speed of light before they are made to collide. The beams travel in opposite directions in separate beam pipes – two tubes kept at ultrahigh vacuum. They are guided around the accelerator ring by a strong magnetic field maintained by superconducting electromagnets. Below a certain characteristic temperature, some materials enter a superconducting state and offer no resistance to the passage of electrical current. The electromagnets in the LHC are therefore chilled to ‑271.3°C (1.9K) – a temperature colder than outer space – to take advantage of this effect. The accelerator is connected to a vast distribution system of liquid helium, which cools the magnets, as well as to other supply services.
What are the main goals of the LHC?
The Standard Model of particle physics – a theory developed in the early 1970s that describes the fundamental particles and their interactions – has precisely predicted a wide variety of phenomena and so far successfully explained almost all experimental results in particle physics.. But the Standard Model is incomplete. It leaves many questions open, which the LHC will help to answer.
- What is the origin of mass? The Standard Model does not explain the origins of mass, nor why some particles are very heavy while others have no mass at all. However, theorists Robert Brout, François Englert and Peter Higgs made a proposal that was to solve this problem. The Brout-Englert-Higgs mechanism gives a mass to particles when they interact with an invisible field, now called the “Higgs field”, which pervades the universe. Particles that interact intensely with the Higgs field are heavy, while those that have feeble interactions are light. In the late 1980s, physicists started the search for the Higgs boson, the particle associated with the Higgs field. In July 2012, CERN announced the discovery of the Higgs boson, which confirmed the Brout-Englert-Higgs mechanism. However, finding it is not the end of the story, and researchers have to study the Higgs boson in detail to measure its properties and pin down its rarer decays.
- Will we discover evidence for supersymmetry? The Standard Model does not offer a unified description of all the fundamental forces, as it remains difficult to construct a theory of gravity similar to those for the other forces. Supersymmetry – a theory that hypothesises the existence of more massive partners of the standard particles we know – could facilitate the unification of fundamental forces.
- What are dark matter and dark energy? The matter we know and that makes up all stars and galaxies only accounts for 4% of the content of the universe. The search is then still open for particles or phenomena responsible for dark matter (23%) and dark energy (73%).
- Why is there far more matter than antimatter in the universe? Matter and antimatter must have been produced in the same amounts at the time of the Big Bang, but from what we have observed so far, our Universe is made only of matter.
- How does the quark-gluon plasma give rise to the particles that constitute the matter of our Universe? For part of each year, the LHC provides collisions between lead ions, recreating conditions similar to those just after the Big Bang. When heavy ions collide at high energies they form for an instant the quark-gluon plasma, a “fireball” of hot and dense matter that can be studied by the experiments.
How was the LHC designed?
Scientists started thinking about the LHC in the early 1980s, when the previous accelerator, the LEP, was not yet running. In December 1994, CERN Council voted to approve the construction of the LHC and in October 1995, the LHC technical design report was published.
Contributions from Japan, the USA, India and other non-Member States accelerated the process and between 1996 and 1998, four experiments (ALICE, ATLAS, CMS and LHCb) received official approval and construction work started on the four sites.
Important figures: the energy of the LHC for Run 2
Quantity |
Number |
Circumference Dipole operating temperature Number of magnets Number of main dipoles Number of main quadrupoles Number of RF cavities Nominal energy, protons Nominal energy, ions Nominal energy, protons collisions No. of bunches per proton beam No. of protons per bunch (at start) Number of turns per second Number of collisions per second |
26 659 m 1.9 K (-271.3°C) 9593 1232 392 8 per beam 6.5 TeV 2.56 TeV/u (energy per nucleon) 13 TeV 2808 1.2 x 1011 11245 1 billion |
What are the detectors at the LHC?
There are nine experiments installed at the LHC: ALICE, ATLAS, CMS, LHCb, LHCf, TOTEM, MoEDAL-MAPP, FASER and SND@LHC. They use detectors to analyse the myriad of particles produced by collisions in the accelerator. These experiments are run by collaborations of scientists from institutes all over the world. Each experiment is distinct, and characterized by its detectors.
What is the data flow from the LHC experiments?
The CERN Data Centre stores more than 30 petabytes of data per year from the LHC experiments, enough to fill about 1.2 million Blu-ray discs, i.e. 250 years of HD video. Over 100 petabytes of data are permanently archived, on tape.
How much does the LHC cost?
- Construction costs (MCHF)
|
Materials |
LHC machine and areas* |
3756 |
CERN share to detectors and detectors areas** |
493 |
LHC computing (CERN share) |
83 |
Total |
4332 |
*This includes: Machine R&D and injectors, tests and pre-operation.
** Contains infrastructure costs (such as caverns and facilities). The total cost of all LHC detectors is about 1500 MCHF
The experimental collaborations are individual entities, funded independently from CERN. CERN is a member of each experiment and contributes to the maintenance and operation budget of the LHC experiments.
- Costs for Run 1
Exploitation costs of the LHC when running (direct and indirect costs) represent about 80% of the CERN annual budget for operation, maintenance, technical stops, repairs and consolidation work in personnel and materials (for machine, injectors, computing, experiments).
The directly allocated resources for the years 2009-2012 were about 1.1 billion CHF.
- Costs for LS1
The cost of the Long Shutdown 1 (22 months) is estimated at 150 Million CHF. The maintenance and upgrade works represent about 100 MCHF for the LHC and 50 MCHF for the accelerator complex without the LHC.
What is the LHC power consumption?
The total power consumption of the LHC (and experiments) is equivalent to 600 GWh per year, with a maximum of 650 GWh in 2012 when the LHC was running at 4 TeV. For Run 2, the estimated power consumption is 750 GWh per year.
The total CERN energy consumption is 1.3 TWh per year while the total electrical energy production in the world is around 20000 TWh, in the European Union 3400 TWh, in France around 500 TWh, and in Geneva canton 3 TWh.
What are the main achievements of the LHC so far?
- 10 September 2008: LHC first beam (see press release)
- 23 November 2009: LHC first collisions (see press release)
- 30 November 2009: world record with beam energy of 1.18 TeV (see press release)
- 16 December 2009: world record with collisions at 2.36 TeV and significant quantities of data recorded (see press release)
- March 2010: first beams at 3.5 TeV (19 March) and first high energy collisions at 7 TeV (30 March) (see press release)
- 8 November 2010: LHC first lead-ion beams (see press release)
- 22 April 2011: LHC sets new world record beam intensity (see press release)
- 5 April 2012: First collisions at 8 TeV (see press release)
- 4 July 2012: Announcement of the discovery of a Higgs-like particle at CERN (see press release)
For more information about the Higgs boson:
The Higgs boson
CERN and the Higgs boson
The Basics of the Higgs boson
How standard is the Higgs boson discovered in 2012?
Higgs update 4 July
- 28 September 2012: Tweet from CERN: "The LHC has reached its target for 2012 by delivering 15 fb-1 (around a million billion collisions) to ATLAS and CMS "
- 14 February 2013: At 7.24 a.m, the last beams for physics were absorbed into the LHC, marking the end of Run 1 and the beginning of the Long Shutdown 1 (see press release)
- 8 October 2013: Physics Nobel prize to François Englert and Peter Higgs “for the theoretical discovery of a mechanism that contributes to our understanding of the origin of mass of subatomic particles, and which recently was confirmed through the discovery of the predicted fundamental particle, by the ATLAS and CMS experiments at CERN’s Large Hadron Collider” (see press release)
See LHC Milestones.
What are the main goals for the second run of the LHC?
The discovery of the Higgs boson was only the first chapter of the LHC story. Indeed, the restart of the machine this year marks the beginning of a new adventure, as it will operate at almost double the energy of its first run. Thanks to the work that has been done during the Long Shutdown 1, the LHC will now be able to produce 13 TeV collisions (6.5 TeV per beam), which will allow physicists to further explore the nature of our Universe.
How long will the LHC run?
The LHC is planned to run over the next 20 years, with several stops scheduled for upgrades and maintenance work.
No. Although powerful for an accelerator, the energy reached in the Large Hadron Collider (LHC) is modest by nature’s standards. Cosmic rays – particles produced by events in outer space – collide with particles in the Earth’s atmosphere at much greater energies than those of the LHC. These cosmic rays have been bombarding the Earth’s atmosphere as well as other astronomical bodies since these bodies were formed, with no harmful consequences. These planets and stars have stayed intact despite these higher energy collisions over billions of years.
Read more about the safety of the LHC here
The High-Luminosity LHC (HL-LHC) is a major upgrade of the Large Hadron Collider (LHC). The LHC collides tiny particles of matter (protons) at an energy of up to 14 TeV in order to study the fundamental components of matter and the forces that bind them together. The High-Luminosity LHC will make it possible to study these in more detail by increasing the number of collisions by a factor of between 5 and 7.5 with respect to the nominal LHC design.
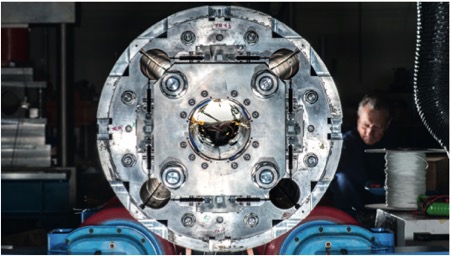
Prototype of a quadrupole magnet for the High-Luminosity LHC. (Image: Robert Hradil, Monika Majer/ProStudio22.ch)
What is luminosity?
Instantaneous Luminosity, which is the measure of the number of potential collisions per surface unit (or cross section) over a given period of time, is an essential indicator of an accelerator’s performance. Integrated luminosity is a measure of the collected data size and defined as the time integral of the instantaneous luminosity measured in inverse femtobarns (fb−1). Assuming the nominal total proton cross section at the LHC at 7 TeV of 100 mbarn, one inverse femtobarn of integrated luminosity equates to 100 million million collisions.
By the end of its first few years of operation at 13 TeV (at the end of 2018), the LHC has produced just above 160 inverse femtobarns of data. The HL-LHC will produce more than 250 inverse femtobarns of data per year and will be capable of collecting up to 4000 inverse femtobarns during its exploitation period of 12 years.
Why High-Luminosity?
The phenomena that physicists are looking for have a very low probability of occurring and this is why a very large amount of data is needed to detect them. Increasing luminosity produces more data, allowing physicists to study known mechanisms in greater detail and observe rare new phenomena that might reveal themselves. For example, the High-Luminosity LHC will produce at least 15 million Higgs bosons per year, compared to around three million collected during LHC operation in 2017.
How will the High-Luminosity LHC work?
Increasing the luminosity means increasing the number of collisions: at least 140 collisions will be produced each time two particle bunches meet at the heart of the ATLAS and CMS detectors, compared to around 40 collision events at present. To achieve this, the beam will need to be more intense and more focused than at present in the LHC. New equipment will need to be installed over about 1.2 of the LHC’s 27 kilometres.
- More powerful focusing magnets and new optics
New, more powerful superconducting quadrupole magnets will be installed on either side of the ATLAS and CMS experiments to focus the particle bunches before they meet. These magnets will be made of a novel superconducting compound, based on niobium and tin [Nb3Sn], used for the first time in an accelerator, which will make it possible to achieve higher magnetic fields than the niobium-titanium alloy used for the current LHC magnets (12 Tesla as opposed to 8). Twenty-four new quadrupole magnets are currently in production, six of which will be installed on each side of the two high luminosity experiments ATLAS and CMS. The use of niobium-tin magnets is also an opportunity to develop this technology for large scale application in future accelerators.
New beam optics (the way the beams are tilted and focused) will notably make it possible to maintain a constant collision rate throughout the time the beams will collide.
- “Crab cavities” for tilting the beams
The particles circulate in the LHC in distinct packages, called bunches. Each bunch is about 30 cm long. This innovative superconducting equipment will give the particle bunches a transverse momentum before they meet, enlarging the overlap area of the two bunches and thus increasing the probability of collisions. A total of sixteen crab cavities will be installed on both side of each of the ATLAS and CMS experiments.
- Reinforced machine protection
As the beams will contain more particles and hence almost twice the stored beam energy of the current LHC, machine protection will need to be reinforced. Around one hundred new, more performant collimators will be installed, replacing or supplementing the existing ones. These devices absorb particles that stray from the beam trajectory and might otherwise damage the machine.
- Crystal collimators
New crystal collimators will be installed in the dedicated cleaning insertions to improve the cleaning efficiency of the insertions for ion beam operation. Without these devices, the higher ion beam intensities would result in unacceptably high losses in the superconducting magnets.
- Innovative superconducting links
Innovative superconducting power lines will connect the power converters to the new accelerator magnets. These cables, which are around one hundred metres long, are made of a novel superconducting material, magnesium di-boride, that is superconducting at a much higher temperature than the magnets, and can therefore be cooled more energy-efficiently by using gaseous Helium. They will be able to carry currents of record intensities, up to 100 000 Amps when being cooled down to temperatures below 50 Kelvin!
- An upgraded accelerator chain
The HL-LHC’s performance will also rely upon the injector chain, i.e. the four machines that pre-accelerate the beams before sending them into the 27-kilometre LHC ring. This accelerator chain has been upgraded during the second long shutdown of the LHC between 2018 and 2021.
What is the work schedule?
In order to install the new equipment and move certain components around, new underground structures and surface buildings are required.
The civil engineering work began in April 2018 at LHC Point 1 (in Meyrin, Switzerland), where the ATLAS experiment is located, and at LHC Point 5 (in Cessy, France), the site of the CMS experiment. A new shaft of around 80 metres, as well as an underground cavern and a 300-metre-long service tunnel has been dug on each site. This service tunnel is linked to the LHC tunnel by four connecting tunnels and 12 vertical cores for each of the two high luminosity experiments ATLAS and CMS. The four main connections between the new and old infrastructures have already been established in 2019. Five surface buildings will be built on each site to house the electrical, cryogenic and cooling and ventilation infrastructure of the new HL-LHC equipment.
In the meantime, the new equipment is being manufactured in Europe, Japan, the United States and China. Canada will also contribute to the production of the state-of-the-art equipment. The experiments are also preparing for major upgrades of their detectors to deal with the deluge of data promised by the HL-LHC.
Installation of the first components (the crystal collimators, beam instrumentation, some other collimators and new shielding) has taken place during the second long shutdown of the LHC. Most of the equipment and the major experiment upgrades will however be installed during Long Shutdown 3, to take place between 2026 and 2028.
How much will the High-Luminosity LHC cost?
The material budget for the accelerator is set at about 1 billion Swiss francs between 2015 and 2028, including many in-kind contributions from international partner laboratories.
Who is involved in the project?
CERN and its Member and Associate Member States are supported by an international collaboration of 43 institutions in 19 countries, including the United States, Canada, Japan and China.
How will society benefit from the HL-LHC?
The HL-LHC will further our fundamental knowledge, which is CERN’s primary mission. To develop the HL-LHC upgrade, CERN is pushing several technologies to new limits, such as electrical engineering, notably in terms of superconductors, vacuum technologies, computing, electronics and even industrial processes. In the long term, these innovations will benefit our daily lives.
For example, superconducting magnets find applications in the fields of medical imaging and cancer treatment with particle beams (hadron therapy). There are also many prospects in the field of electrical engineering: European industry is studying the possibility of using magnesium di-boride cables to transport high electrical power over great distances in a way that is sustainable for the environment.
The HL-LHC project is also contributing to the training of new scientists – physicists, engineers and technicians. Currently, more than 200 bachelor and master students, doctoral students, post-doctoral researchers and fellows of 23 different nationalities are participating in the project.
--
HL-LHC brochure (a printable version of the text shown above)
CERN press release, 15 June 2018: Major work starts to boost the luminosity of the LHC
Is the Large Hadron Collider dangerous?
No. Although powerful for an accelerator, the energy reached in the Large Hadron Collider (LHC) is modest by nature’s standards. Cosmic rays – particles produced by events in outer space – collide with particles in the Earth’s atmosphere at much greater energies than those of the LHC. These cosmic rays have been bombarding the Earth’s atmosphere as well as other astronomical bodies since these bodies were formed, with no harmful consequences. These planets and stars have stayed intact despite these higher energy collisions over billions of years.
Read more about the safety of the LHC here
What happened with the LHC in 2015 and what does CERN plan to do in the future?
The Large Hadron Collider (LHC) restarted at a collision energy of 13 teraelectronvolts (TeV) in June 2015. Throughout September and October 2015, CERN gradually increased the number of collisions, while remaining at the same energy. In November, as with previous LHC runs, the machine run with lead ions instead of protons until mid-December when it had its winter technical stop.
After a successful run in 2016, the most powerful collider in the world was switched back on in spring 2017, followed by a period of tests. After a period of commissioning, the LHC experiments began taking physics data for 2017. Over the coming years, the LHC operators plan to increase the intensity of the beams so that the machine produces a larger number of collisions. This will enable physicists to have a better understanding of fundamental physics.
Why is the Higgs boson referred to as the God particle?
The Higgs boson is the linchpin of the Standard Model of particle physics but experimental physicists weren’t able to observe it until the arrival of the LHC, nearly 50 years after the particle was first postulated. Leon Lederman coined the term ‘the God particle’ in his popular 1993 book ‘The God Particle: If the Universe Is the Answer, What is the Question?’ written with Dick Teresi. In their book, Lederman and Teresi claim the nickname originated because the publisher wouldn’t allow them to call it ‘the Goddamn Particle’ – a name that reflected the difficulty in observing the elusive boson. The name caught on through the media attention it attracted but is disliked by both clerics and scientists.
Is CERN's aim to prove that God does not exist?
No. People from all over the world work together harmoniously at CERN, representing all regions, religions and cultures. CERN exists to understand the mystery of nature for the benefit of humankind. Scientists at CERN use the world’s largest and most complex scientific instruments to study the basic constituents of matter – the fundamental particles. Particles are made to collide together at close to the speed of light. This process gives the physicists clues about how the particles interact, and provides insights into the fundamental laws of nature.
Why does CERN have a statue of Shiva?
The Shiva statue was a gift from India to celebrate its association with CERN, which started in the 1960’s and remains strong today. In the Hindu religion, Lord Shiva practiced Nataraj dance which symbolises Shakti, or life force. This deity was chosen by the Indian government because of a metaphor that was drawn between the cosmic dance of the Nataraj and the modern study of the ‘cosmic dance’ of subatomic particles. India is one of CERN’s associate member states. CERN is a multicultural organisation that welcomes scientists from more than 100 countries and 680 institutions. The Shiva statue is only one of the many statues and art pieces at CERN.
What are the shapes in the CERN logo?
The shapes in CERN’s current logo represent particle accelerators. The logo in this form dates back to 1968, when a decision was made to change the CERN logo from the original one, seen here. Some 114 new designs were proposed, many of which used CERN’s experiments as inspiration. The final design used the original lettering, surrounded by a schematic of a synchrotron, beam lines and particle tracks. Today’s logo is a simplified version of this.
Will CERN open a door to another dimension?
CERN will not open a door to another dimension. If the experiments conducted at the LHC demonstrate the existence of certain particles it could help physicists to test various theories about nature and our Universe, such as the presence of extra dimensions. There is more information here.
What did Stephen Hawking say about Higgs potential destroying the Universe?
Hawking was not discussing the work being done at the LHC.
The LHC observes nature at a fundamental level but does not influence it. Measurements of the Higgs bosonhave allowed us to learn more about the intrinsic nature of the Universe, and it is this that Hawking was discussing. The measured properties of the boson suggest that the Universe is in a quasi-stable equilibrium, though with a lifetime far exceeding anything we can imagine (10100 years). This is explained further in the TEDxCERN talk below:
http://tedxcern.web.cern.ch/video/2013/what-higgs-might-mean-fate-universe
Why does CERN appear in Google Maps when I type certain keywords?
Many of these associations have no grounding in fact, and are a possible result of several users renaming locations on their own maps, keyword searches, or from lots of users creating custom maps, which utilise those search terms.
Can the LHC have an influence on weather patterns and natural phenomena?
No. The magnets at CERN have an electromagnetic field, which is contained with the magnets themselves and therefore cannot influence the Earth’s magnetic field, nor the weather. The strength of the LHC magnets (8.36 teslas) is comparable to the magnetic field found in PET-MRI scanners (up to 9.4 tesla), which are regularly used for brain scans.
Will CERN generate a black hole?
The LHC will not generate black holes in the cosmological sense. However, some theories suggest that the formation of tiny 'quantum' black holes may be possible. The observation of such an event would be thrilling in terms of our understanding of the Universe; and would be perfectly safe. More information is available here.
I saw a video of a strange ritual at CERN, is it real?
No, this video from summer 2016 was a work of fiction showing a contrived scene. CERN does not condone this kind of action, which breaches CERN’s professional guidelines. Those involved were identified and apropriate measures taken.
Does the LHC trigger earthquakes?
The LHC does not trigger earthquakes. Earthquakes are a natural hazard caused by the movement of tectonic plates. As these rigid plates move towards, apart or past each other they can lock up and build up huge stresses at their boundaries, such as the middle of the Atlantic Ocean, or along the Pacific rim. When the plates suddenly slip apart, this stress is relieved, releasing huge amounts of energy and causing an earthquake.
Several million earthquakes occur across the Earth each year but most are too small to be detected without monitoring equipment. There is no means by which the LHC could trigger earthquakes, and no correlation between LHC operation and the occurrence of earthquakes.
Anecdote: Some high precision instruments at CERN are able to detect earthquakes due to their sensitivity to tiny movements. In the LHC, there are more than 100 Hydrostatic Levelling Sensors that monitor the relative displacements of the magnets that steer beams of particles around the LHC’s 27 km ring. These sensors can detect the waves emitted by earthquakes occurring even very far away after their journey through the Earth. Another tool, the Precision Laser Inclinometer, is used to measure the movements of underground structures that can affect the precise positioning of the LHC’s particle detectors. These are also sensitive enough to detect earthquakes.
CERN has a rich educational and cultural programme. As an integral part of this programme, tours of the Laboratory are free of charge.
Find out more about CERN tours via visit.cern, which includes frequently asked questions about CERN tours.